How Precision Breeding Act is advancing gene edited crops
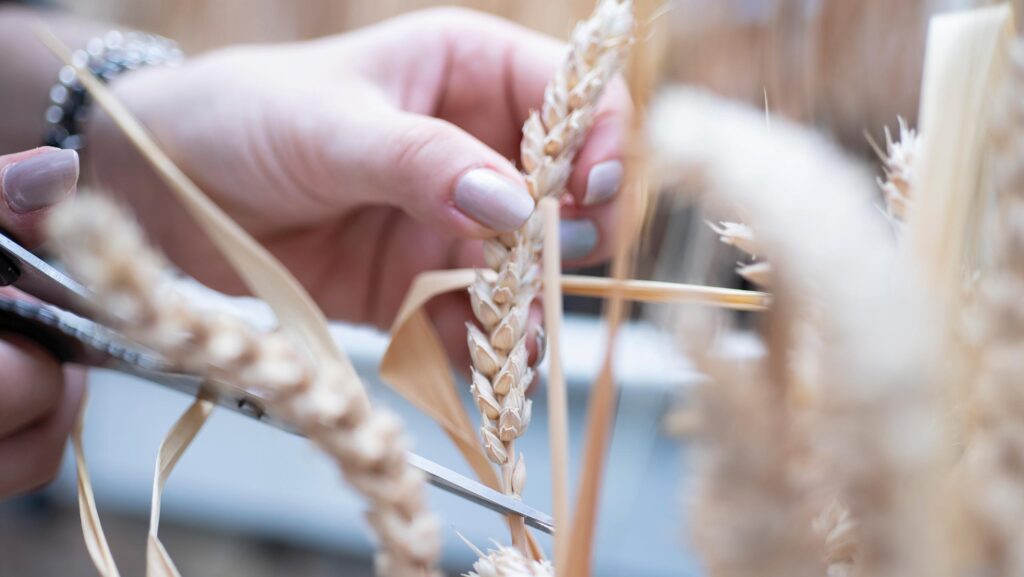
In-field testing of crops that have been gene edited to introduce specific traits and characteristics has become much easier in England following changes to existing legislation on crop biotechnology, with one organisation planning to launch the country’s first on-farm trial next year.
The British On-Farm Innovation Network (Bofin) announced in mid-March that it is planning to multiply enough seed for on-farm trials of gene-edited crops that will take place in the 2025-26 growing season, including low-acrylamide wheat and high-lipid forage crops.
See also: Video: Leicester farm trials weed seed unit on Fendt combine
This has been made possible with the introduction of the Precision Breeding Act, an important step to improve the translation of relevant scientific discoveries into commercial products that are useful for farmers and consumers.
The act means gene edited plants can be grown without needing the same consents as genetically modified (GM) plants, and should mean an increased amount of field testing, and hopefully a quicker pathway to commercialisation.
Currently it takes anywhere from 17 to more than 35 years for a biotechnology solution to go from an idea to having a commercial impact, says Prof Johnathan Napier, who has led the development of camelina as a GM crop producing higher levels of omega-3 oils at Rothamsted Research.
Increased acceptance of gene editing and policy interventions such as the Precision Breeding Act could see that timeline shaved by perhaps five to seven years, he suggests.
“You’re still going to have to do quite a lot of plant breeding in the process, so you’re not going to suddenly go from 35 years to seven weeks.”
But crucially, those ideas that are already at the field trials testing stage potentially have a quicker and easier route to commercialisation.
Low-acrylamide wheat
Among the most advanced is gene edited low-acrylamide wheat, which has just finished year two of field trials in England, becoming the first Crispr/CAS9 gene edited wheat in European trials.
Acrylamide is a toxic chemical that forms during cooking and processing and is regarded as a probable carcinogen in humans.
It can be found in fried, baked or roasted potato and cereal products and coffee, explains Prof Nigel Halford of Rothamsted Research.
“All food businesses have to monitor for acrylamide in their products, so it is already a big deal for the industry,” he says.
The EU Commission has started the process of setting maximum levels, with regulatory enforcement possible for sectors not showing sufficient progress, he adds, with all cereal and fried potato products affected.
“There will be failures at the proposed maximum levels, given the reality of what food businesses can actually achieve,” Nigel says.
For example, industry data for potato crisps shows that potentially about 5% would exceed the proposed maximum level of 1,000 parts per billion, resulting in a product recall.
Potatoes coming out of storage are at more risk of acrylamide formation, and the big gains from improved processing quality control have already been made.
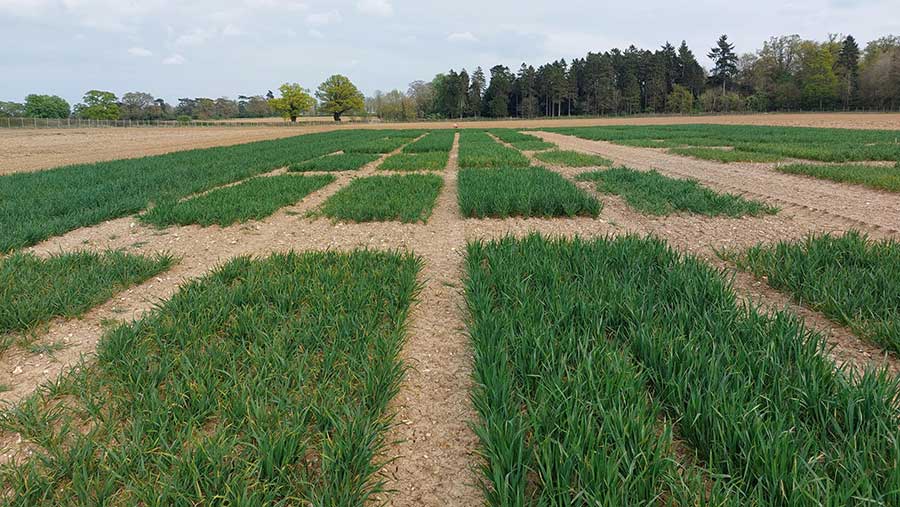
© Rothamsted Research
No similar data has been published for cereal products, Nigel says, but it’s going to be equally difficult for that sector to meet the proposed limits.
Acrylamide is formed when grains, tubers, beans or other vegetable products containing the amino acid asparagine and sugars, such as fructose, glucose and maltose, are heated to high temperatures.
“It’s what gives you the colours, flavours, aromas and textures associated with fried, baked, roasted and toasted foods,” he says.
With a good correlation between acrylamide formation and the amount of free asparagine in wheat flour, Nigel’s research is targeting a set of genes involved in its formation.
In particular, he has found that a particular wheat asparagine synthetase gene, TaASN2, is important, so gene editing has been used to create lines in the wheat variety Cadenza where this gene has been deleted either partially from one of the three wheat genomes, or in all three.
Year one of field trials found a 50% reduction in free asparagine formation where the gene had been knocked out completely. There were no significant effects on yield or grain protein.
Nigel says the reduction translates to a 50% fall in acrylamide formation when flour is heated for 20 minutes.
“Food businesses have said that would enable them to comply with regulations without needing to change other processes or the characteristics of the product,” he says.
The second year of trials included a line in which another asparagine synthetase gene, TaASN1, was knocked out in addition to TaASN2.
Grain from this trial is still being analysed, but the results suggest the level of asparagine is reduced by 90%, and he will investigate how this translates into acrylamide formation in bread and biscuits.
High-energy forage
A second Rothamsted Research-led gene editing programme is investigating how it can be used to produce high-energy forage by increasing the oil content.
Raising the oil content of forage that livestock consume to around 7%, rather than the typical 2% dry weight content of pasture, increases the efficiency of livestock production by providing more metabolisable energy, explains Prof Peter Eastmond, a biochemist at Rothamsted.
“A side product of this is the lipids also suppress methane emissions, potentially by up to 20%,” he says.
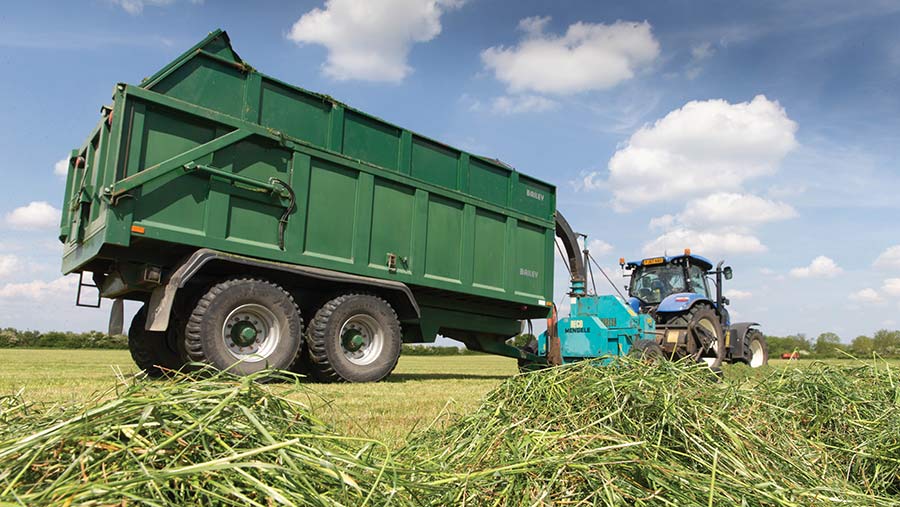
© Tim Scrivener
Increasing the oil content of forage is difficult through conventional breeding as there is relatively little variation in the vegetative tissues of plants, such as roots, stems and leaves.
“Plants can, though, accumulate more oils in their seeds, easily up to 40% of their dry weight, so one way of trying to increase lipid in the vegetative tissues is to try to move this capacity to accumulate oils in seeds to the vegetative tissues,” Peter says.
His research found that a gene which breaks down stored oil when seeds germinate is also present in all other plant tissues, and its activity is a reason why oils do not accumulate in vegetative tissues.
Initial research using a transgenic GM approach, rather than gene editing, showed that by switching off this gene that breaks down oils, and at the same time overexpressing genes that drive the production of fatty acids, oil levels in leaves can be raised to 6-8% – the target for a high-lipid forage trait – in a model plant Arabidopsis.
But because it was a transgenic GM approach – using genetic material from another species – commercialising it in a forage species was tricky, not least because forage grasses outcross with other species easily, he says.
“So I looked at whether you could recreate this trait using gene editing.”
To do so, he has used a technique where, by removing a section of DNA upstream, he essentially tricks the plant into turning on the gene that drives oil accumulation.
“I found that upstream of the gene that can drive oil accumulation in leaves, but isn’t normally switched on, is a gene that is strongly expressed in leaves.
“By using Crispr/CAS9, we were able to delete the intervening section, and use the promoter of the upstream gene to switch on the gene that drives oil accumulation,” Peter explains.
“By doing that you can achieve almost a doubling of the oil content in the leaves.”
Using this technique should be acceptable, as far as he is aware, to the evolving UK regulatory processes, since such deletions can occur by natural processes.
The next hurdle is repeating this work in a useful species rather than Arabidopsis. The ultimate aim is to have the trait in ryegrass, but the team is using barley as a stepping stone, as it is more amenable.
“We’ve already knocked out the lipase gene that breaks down oil in tissues using gene editing and grew these plants in the field,” he says. “This gives about a 1% increase in lipid content in the leaves.”
They have also used a research technique to search for associations between specific genes and lipid content in wheat in mutant-bred populations.
“We’ve been able to find specific mutants that drive an increase in lipid content, which are candidates for a gene editing approach,” Peter says. “We’re hoping to combine this with disruption of breakdown.”
As with the low-acrylamide wheat, the ideal next step is to grow the barley on a field scale in the UK and feed to cattle, he says. “I’m also building collaborations to help translate this work into ryegrass.”
Gene-edited break or cover crops
Gene editing could help transform an ancient legume crop – which has one very serious drawback – into a potential alternative break or cover crop for UK growers.
Grass peas have been cultivated globally – but not in the UK – for about 8,000 years, after first being domesticated in the Balkans, according to Dr Peter Emmrich, who leads research into the crop for the John Innes Centre and the Norwich Institute for Sustainable Development.
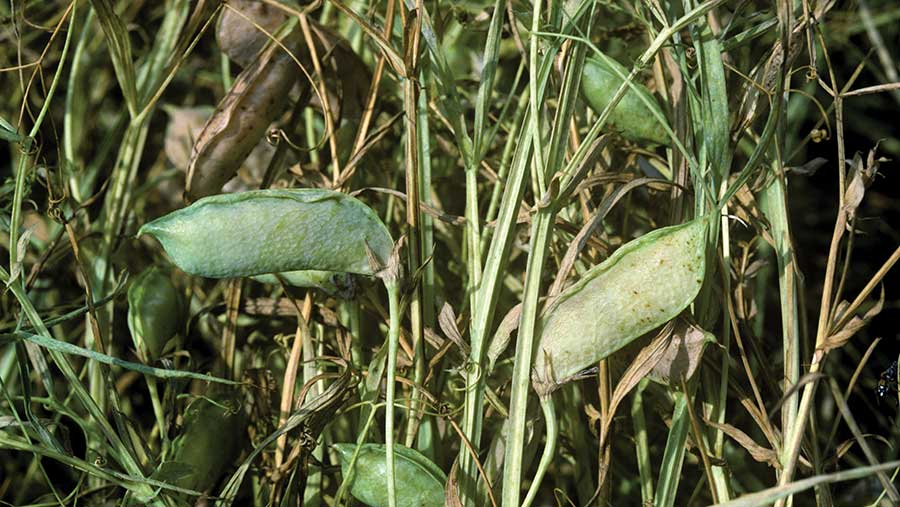
Grass peas © Nigel Cattlin/AlamyStock Photo
“It’s widely grown in South Asia, parts of Ethiopia, Eritrea, northern Africa and southern Europe for human consumption, and more recently as cover crops or for animal feed in Australia, China and the Americas,” he says.
The drawback is that the peas, which look a bit like chickpeas, can be toxic to both humans and animals, potentially causing paralysis.
“It’s typically only if you are heavily malnourished and rely on grass peas as a staple food,” Peter stresses. “Which is why people have still been using it as a food for thousands of years.”
Nonetheless, its toxicity represents a barrier to its wider adoption, which is why Peter and a team at the John Innes Centre are researching how to reduce the toxin production.
There are already varieties of grass pea bred traditionally with reduced toxin production, Peter says, while lines bred using mutagenesis at the John Innes Centre produce about 90% less toxin.
“But we don’t know the genetic basis of why that is happening yet.”
A gene editing approach, developed by project partners at the University of Queensland in Australia, is also showing promise.
“Technically it is very challenging to make gene editing work in legumes, but they have achieved it and we are now working on multiple ways to make it more efficient to both resolve the toxin problem and to improve grass peas in many other ways.”
An annual crop, its tolerance of drought, flood and salinity potentially makes grass peas an interesting opportunity for UK growers, he suggests.
“It’s also highly nutritious, very high in protein, so it is useful forage as long as you manage the toxicity risk.”
Research scientist Dr Anne Edwards grew test plots of grass peas on the John Innes Centre farm this season. Planted in May, it was harvested in October although seed was produced from July, she says.
“It does perfectly well here and from our comparisons we think it could yield similarly to a typical combining pea crop.”
That could make it very interesting as an alternative break crop, particularly with greater tolerance to climate stress, the team thinks, although more work is needed to breed locally suited varieties and to build a market for the product.
Camelina
Gene editing is also being used to improve the potential of an oilseed crop, camelina, which has already been genetically modified to produce omega-3 fish oils.
The original work to transform camelina was carried out by Prof Johnathan Napier at Rothamsted.
He recognised that fish are rich in fish oils because of their diet, rather than because they produce the oils, and with the amount of fish oil being fed to farmed fish reducing due to expense and declining natural resources, there is a need for a new, sustainable source of omega-3 fish oils.
That led to a simple idea: take the genes from the algae that produce the fish oils and are the base of the food web and put them into land-based plants.
“The fact that I’m still talking about this 25 years later confirms it was more difficult than we initially thought,” he jokes.
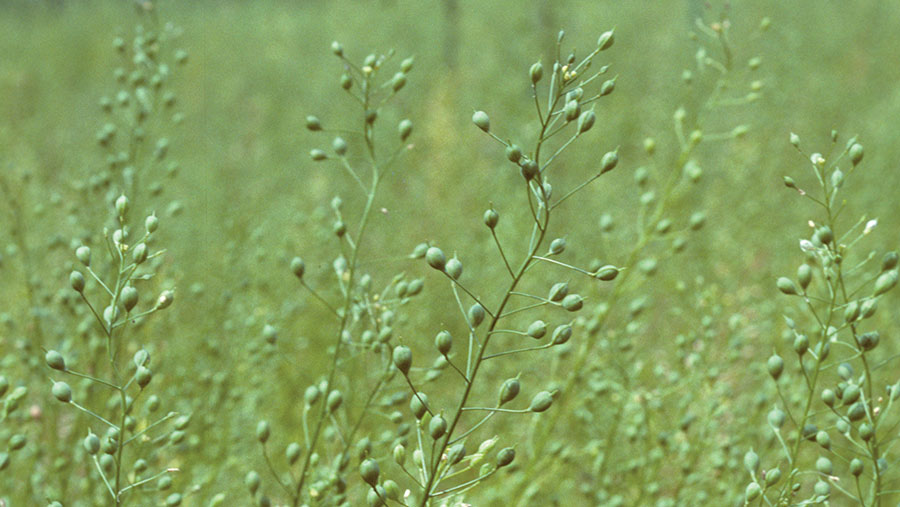
Camelina © Nigel Cattlin/Alamy Stock Photo
However difficult, it has now been successfully achieved using transgenic techniques which means the plant makes two fish oils, eicosapentaenoic acid (EPA) and docosahexaenoic acid (DHA).
The process has since been optimised using gene editing to improve the accumulation of the oils in the seed.
One method has been to knock out some genes that divert precursors of the oils to be made into complex lipids rather than the targeted fish oils, explains Dr Richard Haslam, a senior research scientist at Rothamsted.
“When we analyse the seed we get increased production of DHA,” he says.
Research is now looking at whether more complex gene edits can further improve the oil production, but the crop is already on the cusp of commercialisation.
Agreements have been signed with US agricultural biotech company Yield10 and global aquafeed producer BioMar Group to commercialise GM camelina to produce omega-3 oil as a high-quality supplement to the scarce supply of marine long-chain fatty acids used in aquafeed.
Initial ramping up of seed production of camelina lines with the trait have begun in US and Chile, with potential for the crop to be grown both as a cash and a cover crop.
Gene editing solution for virus yellows?
Combining two technologies – gene editing and RNA interference, also known as gene silencing – could offer a way to control virus yellows in sugar beet.
British Sugar has invested in a collaboration with Norwich-based biotech company Tropic to explore how Tropic’s Gene Editing Induced Gene Silencing (GEiGS) platform can leverage the crop’s own natural defence mechanism.
It works by exploiting how protein production can be disrupted through RNA interference.
Messenger RNA is transcribed from protein-coding DNA to direct protein production, but it can interfered with by “small RNAs” produced from non-coding genes within an organism’s genome.
The small RNAs have a similarity to a specific messenger RNA and effectively degrade it so the target protein is not produced – a process called RNA interference.
By using gene editing, Tropic’s platform makes small edits to a non-coding gene to redirect the resulting small RNA’s activity to a new target gene.
The plan is to use this technology to target genes belonging to the virus to silence them and prevent infection.
Once GEiGS designs are generated they can be passed to plant breeders, allowing them to integrate virus yellows resistance traits into sugar beet varieties, with an expectation this will take five to eight years.